Influence of structure on the magnetoelectric coupling of SrFe12O19 – BNT-BT composites
Project Director: Dr. Simona GRECULEASA
Acronym: StructMagnetoelComp
Project Code: PN-III-P1-1.1.-PD-2019-0724
Contract number: PD 130/2020
Project Director: Dr. Simona Gabriela Greculeasa
Mentor: Dr. Valentin Serban Teodorescu
Project Type: National
Programme:
Program 2 –Cresterea competitivitatii economiei romanesti prin cercetare,dezvoltare si inovare. Subprogramul 2.1 - Competitivitate prin cercetare, dezvoltare si inovare, Proiect experimental-demonstrativ
Financed by: Unitatea Executiva pentru Finantarea Invatamantului Superior, a Cercetarii, Dezvoltarii si Inovarii, UEFISCDI
Contractor: National Institute for Materials Physics
Status: Finalized
Start date: August 18th 2020
Final date: December 17th 2021
Summary
Composites based on M-type hexaferrites and lead-free ferroelectrics
are in the spotlight of the research community as promising
multiferroic heterostructures. In this respect, this project proposes a
complex analysis of a composite formed of SrFe12O19 and
(Bi1/2Na1/2)TiO3-BaTiO3 (BNT-BT) in order to give more insight into
the link between structural aspects and magnetoelectric properties.
The Sr hexaferrites and BNT-BT powders will be prepared by the
sol-gel method, and further processed by spark plasma sintering (SPS)
method. The morphology and structure will be investigated by X-ray
diffraction (Rietveld refinement) and electron microscopy (SEM, TEM),
while the global electric and magnetic properties will be studied by
ferrotester method and magnetometry (SQUID). The local structure
will be analyzed in detail by 57Fe Mössbauer spectroscopy. Direct
magnetoelectric coupling will be investigated in the case of the
multiferroic composite via lock-in technique.
Project director: Greculeasa Simona-Gabriela
Mentor: Teodorescu Valentin Serban
Scientifical Report
In the present report, composites based on strontium hexaferrite (SrFe12O19, SFO) and bismuth sodium titanate - barium titanate (0.92(Bi0.5Na0.5TiO3) – 0.08 (BaTiO3), BNT-BT) were investigated. The samples were prepared by sol-gel (citrate method), varying the BNT-BT quantity with x = 0 (S1), 0.2 (S2), 0.5 (S3), 0.8 (S4), 1 (S5). The structural, morphological and local structure investigations were performed by X-ray diffraction X (XRD), electron microscopy (SEM-EDS, HR-TEM) and Mössbauer spectroscopy.
Figure 1 shows the diffraction patterns of the composites, while Rietveld refinement was performed with MAUD program [1]. The main phases are the desired ones, the SFO magnetoplumbite hexagonal structure (space group P63/mmc; a=5.886 Å, c=23.066 Å) and BNT trigonal perovskite-type structure (space group R3c:H; a=5.46 Å; c=3.915 Å), respectively. The BaTiO3 contribution is considerably reduced; however the broadening of the diffraction lines indicate the diffusion of the Ba titanate in the Bi-Na titanate lattice to form a solid solution. A secondary hematite (α-Fe2O3) of trigonal structure (space group R-3c:R; a=5.426 Å; α=54.587), is also present, especially in the cases of S3 and S4 samples.
Figure 1 XRD patterns of samples: S1-S5.
Figure 2. SEM-SE images of samples S1 (a,b), S2 (c,d) și S5 (e,f) at different magn.: 5k (left) și 40k (right).
The SEM images, as well as the elemental distribution mapping images of the composites are shown in figures 2 and 3. In the case of the SFO sample (S1), an irregular morphology and rough texture is observed. Sample S2, the composite with x=0.2, presents a morphology of type hexagonal prism. The sample PMN-PT (S5) is showing a sponge-like morphology.
Figure 3. (a) SEM-SE and EDS (b) images of sample S1. (c) SEM-SE and EDS (d) images of sample S2. SEM-SE image (e) and EDS images of sample S5: Bi (f), Na (g), Ti (h) and O (i).
Figure 4. Sample S2: HR-TEM image (a), TEM image of a SFO particle (b) and corresponding SAED pattern (c). TEM-BF image (d) and EELS for Fe (e), O (f) and Sr (g).
In the case of transmission electron microscopy, the S2 sample was investigated diffraction contrast (TEM, SAED), as well as in phase contrast (HR-TEM). Also, the elemental mapping was performed using the EEL spectrum. In the HR-TEM image, the presence of the secondary phase of hematite α-Fe2O3, is evidenced. In the SAED pattern and in the EELS mapping, the formation of the hexagonal SFO is evidenced, without the ferroelectric. However, the presence of both phases is supported by XRD and EDS results.
The local configuration of the magnetic component of the composites was determined by Mössbauer spectroscopy. The spectra of samples S2 and S3 are shown in figure 5, and the hyperfine parameters, as well as the relative areas of the sublattices, are presented in table 1.
Figure 5. Mössbauer spectra of the composites: S2 (left) și S3 (right).
Table 1. Main features and hyperfine parameters of the composites: linewidth (Γ), isomer shift (IS), quadrupole splitting (QS), magnetic hyperfine field (Bhf) and relative areas of the components (RA).
Sample | Phase | Component | Orient. | Coord. | Γ (mm/s) | IS (mm/s) | QS (mm/s) | Bhf (T) | RA (%) |
S2 | SFO | 4f2 | ↓ | oct. | 0.37 | 0.38 | 0.27 | 52.4 | 13 |
2a | ↑ | oct. | 0.34 | 0.33 | 0.15 | 51.0 | 13 | ||
4f1 | ↓ | tetr. | 0.38 | 0.27 | 0.2 | 49.1 | 18 | ||
12k | ↑ | oct. | 0.54 | 0.34 | 0.39 | 42.0 | 51 | ||
2b | ↑ | fivefold | 0.3 | 0.22 | 2.11 | 40.7 | 5 | ||
S3 | SFO | 4f2 | ↓ | oct. | 0.27 | 0.4 | -0.07 | 53.8 | 9 |
2a | ↑ | oct. | 0.29 | 0.38 | -0.18 | 52.2 | 26 | ||
4f1 | ↓ | tetr. | 0.6 | 0.34 | 0.18 | 49.6 | 26 | ||
12k | ↑ | oct. | 0.76 | 0.35 | 0.41 | 42 | 33 | ||
2b | ↑ | fivefold | 0.3 | 0.64 | 2.8 | 40.4 | 3 | ||
α-Fe2O3 | SP | - | - | 0.4 | 0.29 | 0.5 | - | 3 |
Strontium hexaferrite is a ferrimagnet with magnetoplumbite structure and the magnetic properties are given by the superexchange interaction (Fe3+–O–Fe3+). Five Wyckoff crystallographic positions of the Fe3+ ions are present, evidenced in the five magnetic sextet sublattices of the Mössbauer specta. The hyperfine parameters shown in table 1 are in accordance with other studies [2-4]. The secondary hematite phase was assigned to the central superparamagnetic (SP) doublet.
In conclusion, SrFe12O19 - x BNT-BT (x=0; 0.2; 0.5; 0.8; 1) composites were prepared by sol-gel. The structure of the composites was probed by X-ray diffraction, confirming the presence of the involved phases. The morphology and structure was determined by electron microscopy (scaning, transmission). The local structure was also probed by Mössbauer spectroscopy and different positions of the iron ions were evidenced in the magnetic component.
Referenes
[1] L. Lutterotti, M. Bortolotti, G. Ischia, I. Lonardelli, H.-R. Wenk, Z. Kristallogr., Suppl. 26 (2007) 125-130.
[2] S.K. Chawla, P. Kaur, R.K. Mudsainiyan, S.S. Meena, S.M. Yusuf, Journal of Superconductivity and Novel Magnetism 28 (2015) 1589-1599.
[3] A. Vijayalakshmi, N.S. Gajbhiye, Journal of Applied Physics 83 (1998) 400.
[4] B.J. Evans, F. Grandjean, A.P. Lilot, R.H. Vogel, A. Gerard, Journal of Magnetism and Magnetic Materials 67 (1987) 123-129.
Scientifical and Technical Report in extenso
Summary of stage 2
Novel SrFe12O19 –BNT-BT nanocomposites were explored in this report. A first set of samples were produced by sol-gel method and compacted by conventional sintering. The composition, morphology, local structure, dielectric and magnetic properties were investigated by X-ray diffraction, Transmission Electron Microscopy, Impedance Analysis, Mössbauer spectroscopy, and SQUID magnetometry. The desired composition and the presence of the magnetoplumbite SrFe12O19 and perovskite BNT-BT structures were verified by X-ray diffraction. Irregular morphology and large size distributions are evidenced in the electron microscopy micrographs. The reported room temperature dielectric constants in this study are the highest values obtained in multiferroic composites at room temperature: giant dielectric constants (~ 1.3 * 106) were obtained, relative to 0.13 * 104 in BNT-BT. The hyperfine parameters allowed the identification of the Wyckoff positions of the Fe ions corresponding closely to the theoretical case. The hard magnetic character of the SrFe12O19 phase is evidenced from the magnetic measurements. For the first time in multiferroic composites, superdielectric characteristics are evidenced at room temperature.
For the second set of samples, the dielectric, electric and magnetoelectric properties of SrFe12O19 – BNT-BT0.08 nanocomposites were explored. The desired composition and the existence of the magnetoplumbite SrFe12O19 and perovskite BNT-BT structures were verified by X-ray diffraction. The dielectric constant values approached the case of BNT-BT due to the small amount of hexaferrite content. The electric and the magnetoelectric properties were derived.
Scientifical and Technical Description
Introduction
Multiferroic nanomaterials are key candidates for various applications such as magnetic field sensors, microwave devices, magnetic recording read heads, Random access memory (RAM) devices, Photovoltaic multiferroic solar cells, energy harvesting, multicaloric refrigeration, magnetoelectric spin-orbit logic (MESO) devices, and bio-medical applications (drug delivery, tissue engineering etc.) [1-4]. Relative to single phase multifferoics, joining magnetic materials of high permeability and superior ferroelectric materials leads to formation of composites with superior performances.
Taking into account ongoing efforts to remove lead from the industry due to poisoning, which has drastic effects on health (e.g. of industrial workers) and environment [5], promising lead-free magnetoelectric composites are represented by hexaferrites and perovskites. An extensive combinatorial study of the composite SrFe12O19 − BaTiO3 polycrystalline strips with a gradient composition was performed by R. Pullar [6] regarding dual ferroic properties. A low content of hexaferrite is preferred to avoid increasing conductivity, which is expected to inhibit any ferroelectricity and magnetoelectric coupling. In BaTiO3-BaFe12O19 composites, dual ferroic properties were investigated in references [7,8], while the local piezoelectric coefficient and polarization switching parameters were found to change on the application of an external magnetic field in [9]. After doping the Ba titanate with Sr, in BaFe12O19–Ba0.5Sr0.05TiO3 heterostructured thick films [10], prospects for mm-range microwave devices were envisaged. In 0.7BaTiO3–0.3SrFe12O19 [11], a small positive magnetodielectric effect was detected at lower temperatures and a large negative and positive MD induced at a resonance frequency was observed at temperatures higher than 150 K. The material (Bi1/2Na1/2)TiO3-BaTiO3 (BNT-BT) has superior piezoelectric properties relative to BT [12, 13]. In this respect, a lead-free composite such as BNT-BT – SrFe12O19 is expected to be a promising multiferroic heterostructure.
Also, the energy density of capacitors can be improved either by increasing the specific surface area or by increasing significantly the dielectric constant. However, in the former case, performances are not enough adequate even in theoretical graphene based supercapacitors. Therefore, many efforts have been taken to improve significantly the dielectric constant of materials. The discovery of high dielectric constants (εr ~ 104) in CaCu3Ti4O12 (CCTO) [14] more than two decades ago and, for example, in multiferroic composites such as 0.9 SrFe12O19 – 0.1 BaTiO3 [6], BaTiO3–BaFe12-xMnxO19 [15], and (Bi0.5Na0.5TiO3 –BaTiO3)– CoFe2O4 [16] paved the way for the design of colossal dielectric materials as potential candidates for microelectronics, energy storage and power electronics integration [17,18]. Collosal dielectric constants (εr > 105) were further obtained in CCTO based materials [19,20] and in perovskites and double perovskites, some with different substitutions: BaZr0.02(Fe0.5Nb0.5)0.98O3 ceramics optimized by sintering conditions [21], LaFeO3 [22] and La2-xBixCoMnO6 [23], and doped rutile: (Nb2O5-Dy2O3-SiO2) Co-doped TiO2 [24] and (TaxSm1-x)0.04Ti0·96O2 [25], (Sb5+, Nb5+) and (Sm3+, Y3+) co-doped Ti0.9Zr0.1O2 [26]. In addition, the dielectric constant is expected to increase even further when an external magnetic field is applied [11]. A new class of materials is represented by superdielectric materials (SDMs) that have dielectric constants many orders of magnitudes higher relative to standard Ba titanate dielectrics, up to 1011. Superior dielectric properties were obtained for the following SDMs: (Nb+Al) codoped TiO2 ceramics (εr ~ 106) [27], La2-xYx-y BayNiO4 (εr ~ 107 at 105 Hz) [28], LaFe1−xZnxO3−δ (εr ~ 108) [29], Bi1-x(Sr0·5Pb0.5)xFeO3 (εr ~ 106) [30], and (Zn0.91Mn0.03Co0.06O)x / Cu0.5Tl0.5Ba2Ca2Cu3O10-δ diluted magnetic semiconductor / superconductor composites (εr ~ 108) [31]. However, the highest dielectric constants were obtained not in solid medium, but in SDMs based on dispersed liquids containing dissolved salts, such as alumina/boric acid solution (εr ~ 1.8 * 109) [17], nylon fabrics saturated with aqueous NaCl solutions (εr ~ 1011) [32] and fumed silica/aqueous NaCl (εr ~ 1011) [33].
Regarding multiferroic composites, relatively high dielectric constants were obtained in a few materials only at high temperatures, such as the case of La doped BiFeO3-PbTiO3 composite (7*104 at 493 K) [34], and Fe nanocrystals embedded into an epitaxial BaTiO3 (BTO) matrix (106 at 593 K and < 103 at 293 K) [35].
In this report, electric, dielectric, magnetic and magnetoelectric properties of (1-x) SrFe12O19 – x BNT-BT composites were analyzed with respect to the structural and morphological properties.
The preparation conditions were optimized relative to the first stage of the project. The first set of samples, (1-x) SrFe12O19 – x (0.93 Bi0.5Na0.5TiO3 – 0.07 BaTiO3) composites, where x=0 (sample denoted as A), x=0.5 (sample B), x=0.8 (sample C) and x=1 (sample D), respectively, were prepared by the sol-gel method. The resulting powders were calcinated at 700°C for 2h and further sintered at 1160 °C for 15 minutes. Subsequently, the ceramic disks were coated with silver paste electrodes, cured at 200°C for 1 h.
A second set of composite sample (sample E) was prepared by a method similar to [36]. A core-shell like sample were obtained.
The X-ray diffraction (XRD) measurements were performed by Bruker D8 Advance X-Ray Diffractometer. The MAUD software was used for the Rietveld refinement [37]. Transmission Electron Microscopy analysis is performed by Jeol AFM200F. Dielectric measurements of the sintered ceramic samples were performed using an AGILENT 4294 A Precision Impedance Analyzer (Agilent Technologies, Santa Clara, CA). The electric properties were investigated by a ferrotester, using a triangular voltage signal. The local structure was investigated by 57Fe transmission Mössbauer spectroscopy. The Mössbauer spectra were acquired at room temperature with a constant acceleration spectrometer and using a 57Co(Rh) source. The NORMOS Software [38] was used for the least-squares fitting of the Mössbauer spectra. The values of the isomer shift were reported relative to α-Fe at room temperature. The magnetic hysteresis loops were investigated using a Quantum Design MPMS–5S SQUID magnetometer using the Reciprocal sample option (RSO). The magnetoelectric properties were measured by a homemade device based on lockin amplifier.
Results
Sample Set 1
Structural and morphological properties
Figure 1 shows the diffraction patterns of the samples SrFe12O19 and BNT-BT, as well as of the composites SrFe12O19 – BNT-BT, and the results derived from the Rietveld refinement are listed in table 1. Only the hexagonal magnetoplumbite SrFe12O19 phase was evidenced in the XRD pattern of sample A. Main features of the perovskite Bi0.5Na0.5TiO3 phase were evidenced in sample D. Slight broadening of the lines is due to the small contribution of the BaTiO3 lattice. The desired ratio of the components are roughly obtained. Crystallite size of hexaferite decreases at half when in composite, while the crystallite size of the titanate does not change significantly.
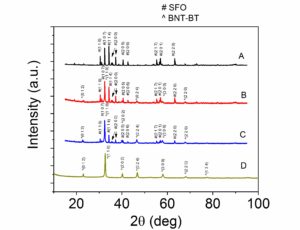
Figure 1. XRD patterns of the samples: A (a), B (b), C (c), and D (d).
Table 1 Molar compositions, crystallographic data and main structure Rietveld refinement parameters.
Sample | Contrib. (% mol.) | aSFO (Å) | cSFO (Å) | aBNT (Å) | cBNT (Å) | dSFO (nm) | dBNT (nm) | ||
SFO | BNT | ||||||||
A | 100 | - | 5.8803 | 23.053 | - | - | 315 | - | |
B | 55 | 45 | 5.88019 | 23.0493 | 5.52331 | 3.91763 | 176 | 38 | |
C | 23 | 77 | 5.88074 | 23.0428 | 5.51673 | 3.91982 | 125 | 38 | |
D | - | 100 | 5.51474 | 3.91674 | 41 | ||||
The Transmission Electron Microscopy micrographs, as well as the Energy Dispersive X-ray System maps, of the composite samples, are shown in Figure 2. Irregular morphology and crystallinity are evidenced. The particle size distribution is rather large. The presence of the involved elements is confirmed by the EDS maps.
Figure 2. TEM micrographs (a), SAED patterns (b) and elemental maps (c-e) of the samples A and D. TEM micrographs (a,b) and SAED patterns of the sample B. TEM micrographs (a), SAED patterns (b,c) and elemental maps (d-i) of the sample C.
Electric and dielectric properties
Figure 3. Electric properties of sample D (a). Dielectric constants (b, d) and losses (c) of samples B, C and D.
The electric hysteresis of sample D is shown in figure 3 (a). The parameters of the electric hysteresis are: saturation polarization of 17 μC/cm2, remanent polarization of 11 μC/cm2, and electric coervice field of 13 kV/cm.
The dielectric properties of the composite samples were studied by measuring the capacitance and dielectric loss, at different frequencies between 0.9 kHz and 1 MGHz. The dielectric constant and dielectric loss of samples B, C and D, were represented in figure 3 (b) and (c). Giant dielectric constant values were obtained at room temperature, up to 1.3 * 106 at 0.9 kHz and 7*104 at 1 MHz for sample B, containing more SrFe12O19 relative to sample C. At very low frequency, of 0.01 Hz, the dielectric constant reaches 1.9 * 106 (figure 3 d). These values are the highest in this type of multiferroic composites, well above the dielectric constants corresponding to typical ferroelectrics such as BaTiO3 [39] in the order of 103. Only a handful SDMs surpass the maximum value obtained in this study at room temperature, such as La2-xYx-y BayNiO4, LaFe1−xZnxO3−δ, and (Zn0.91Mn0.03Co0.06O)x / Cu0.5Tl0.5Ba2Ca2Cu3O10-δ [28,29, 31] and liquids containing dissolved salts [17, 32, 33]. High dielectric losses, of the order of 101, were obtained, similar to [40,41], also being 1 or even 2 orders of magnitude lower relative to other studies [42,43]. Therefore, SDMs are not quite ready for applications and efforts need to be made to optimize the preparation conditions and lower the dielectric losses in order to move from fundamental science to practical dielectric devices [44]. The Hakki Coleman method was not suitable to apply due to the large dielectric loss values and only impedance spectroscopy was used.
Local structure properties
The strontium hexaferrite is a ferrimagnet having the magnetoplumbite structure and its magnetic properties are governed by superexchange interaction (Fe3+–O–Fe3+). Five different crystallographic positions of Fe ions are present: 12k, 2a and 4f2 are octahedral, 4f1 is tetrahedral, while 2b is a site in which the ferric ion is surrounded by five oxygen atoms, forming a trigonal bipyramid (2b) [45].
Figure 4. Mössbauer spectra of the composites: A (a), B (b), and C (c).
The local structure of the (1-x) SrFe12O19 – x BNT-BT composites is evidenced in the Mössbauer spectra shown in figure 4. Table 3 presents the hyperfine parameters that were used to identify the five positions characteristic of the iron ions in the hexaferrite. Ferric (Fe3+) iron ions are confirmed by the isomer shift values of all five sublattices, found in the range 0.27 -0.4 mm/s. Relatively reduced values are obtained for the quadrupole splitting, except for the sublattice corresponding to the 2b site, due to its strongly distorted environment. Considering the magnetic hyperfine field values, the five magnetic sublattices are assigned to the crystallographic positions in the following order (decreasing Bhf): 4f2, 2a, 4f1, 12k, and 2b, respectively, similar to [46,47]. Within experimental errors, the contribution of the different iron phases is similar to the theoretical one.
Table 3. Hyperfine parameters of the composites: linewidth, isomer shift IS, quadrupole splitting QS, hyperfine magnetic field Bhf, relative area of the sublattices in the spectrum RA (with experimental errors, Er (RA)), compared to the theoretical relative area of the components, RA,th.
Phase | Sublattice | Γ (mm/s) | IS (mm/s) | QS (mm/s) | Bhf (T) | RA (%) | Er (RA) | RA,th (%) |
A | 4f2 | 0.37 | 0.37 | 0.26 | 52.9 | 18 | 2 | 16.7 |
2a | 0.28 | 0.34 | 0.15 | 51.7 | 9 | 2 | 8.3 | |
4f1 | 0.35 | 0.26 | 0.16 | 49.9 | 18 | 2 | 16.7 | |
12k | 0.38 | 0.33 | 0.38 | 42.1 | 49 | 1 | 50 | |
2b | 0.3 | 0.28 | 2.27 | 41.5 | 6 | 1 | 8.3 | |
B | 4f2 | 0.44 | 0.4 | 0.3 | 52.7 | 17 | 2 | 16.7 |
2a | 0.27 | 0.33 | 0.13 | 51.9 | 9 | 1 | 8.3 | |
4f1 | 0.36 | 0.27 | 0.17 | 49.9 | 17 | 2 | 16.7 | |
12k | 0.38 | 0.33 | 0.37 | 41.9 | 50 | 1 | 50 | |
2b | 0.36 | 0.3 | 2.28 | 41.6 | 7 | 1 | 8.3 | |
C | 4f2 | 0.45 | 0.39 | 0.29 | 52.8 | 17 | 2 | 16.7 |
2a | 0.28 | 0.34 | 0.14 | 51.8 | 10 | 2 | 8.3 | |
4f1 | 0.34 | 0.27 | 0.16 | 49.8 | 18 | 2 | 16.7 | |
12k | 0.36 | 0.33 | 0.38 | 41.8 | 49 | 1 | 50 | |
2b | 0.38 | 0.31 | 2.3 | 41.4 | 6 | 1 | 8.3 |
Magnetic properties
The magnetic properties of the samples A, B and C are derived from the hysteresis curves (figure 5), while the magnetic parameters are introduced in table 3. The hard magnetic character of SrFe12O19 is evidenced by the high values of the coercive field. The saturation magnetization values in strict relation to the weight percent of the SrFe12O19 phase in the composite.
Figure 5. Magnetic hysteresis curves of the samples. The lines connecting the experimental points are for guidance.
Table 3. Magnetic parameters of the samples: coercive field HC, remanent magnetization MR, saturation magnetization MS, squareness ratio MR / MS.
Sample | HC (Oe) | MR (emu/g) | MS (emu/g) | MR / MS |
A | 1931 | 29 | 67 | 0.43 |
B | 1835 | 24 | 54 | 0.44 |
C | 2381 | 19 | 38 | 0.5 |
Sample Set 2
Structural properties
Regarding the second set of sample, figure 6 shows the diffraction patterns of the sample E and the results derived from Rietveld refinement are listed in table 4. The hexagonal magnetoplumbite SrFe12O19 phase (space group P63/mmc) and the perovskite BNT-BT phase (space group P4bm) were evidenced in the XRD patterns. The desired ratio of the components are roughly obtained. The crystallite size of the SFO phase is 50% higher relative to the BNT-BT phase.
Figure 6. XRD patterns of the composite samples.
Table 4 Molar compositions, crystallographic data and structure Rietveld refinement parameters.
Sample | Contrib. (% mol.) | aSFO (Å) | cSFO (Å) | aBNT-BT (Å) | cBNT-BT (Å) | dSFO (nm) | dBNT-BT (nm) | |
SFO | BNT-BT | |||||||
E | 4 | 96 | 5.8822 | 23.0625 | 5.5166 | 3.9176 | 104.8 | 69.8 |
Dielectric properties
Figure 7. Dielectric constant and loss of sample E.
The dielectric properties of the composite sample E were studied by measuring the capacitance and dielectric loss, at different frequencies between 0.9 kHz and 1 MGHz. They are represented in figure 7. The dielectric constant values, roughtly of the order of 103 at low frequencies, are similar to BNT-BT samples [36], due to the small amount of the SrFe12O19 contribution. The dielectric loss values are relatively low, suitable for dielectric devices.
Electric and magnetoelectric properties
The electric hysteresis of sample E is shown in figure 8. The remanent hysteresis was extracted from the total hysteresis. The parameters of the hysteresis are as follows: saturation polarization of 5 μC/cm2, remanent polarization of 3.6 μC/cm2 and coercive field of 1500 V/mm/s.
The magnetoelectric properties of the samples are evidenced in figure 9. The presence of the magnetoelectric coupling is probed by the variation of Vrms with magnetic field and also with the shift in the phase hysteresis.
Figure 8. Electric hysteresis of sample E.
Figure 9. Magnetoelectric properties of the sample E: Vrms (a), phase (b).
Conclusions
All of the objectives of the stage were fulfilled. The preparation conditions were optimized relative to the first stage of the project. The structural, morphological, electric, dielectric, magnetic and magnetoelectric properties were analyzed in detail.
In summary, (1-x) SrFe12O19 – x BNT-BT0.08 nanocomposites were prepared by sol-gel synthesis and processed by conventional sintering. The involved structures, lattice parameters and crystallite sizes of the components and their contribution are revealed by XRD. From the TEM results, the samples show irregular morphology and high size dispersion. The frequency dependence of the dielectric properties was investigated in the range 0.9 kHz – 1 MHz. Giant dielectric constants were obtained for the multiferroic composites, as much as 1.3 * 106. The Mössbauer spectroscopy results revealed the different Wyckoff positions in the magnetoplumbite structure, close to the theoretical case. The magnetic hysteresis loops showed the hard magnetic character of the SFO phase.
Further studies are envisaged to give more insight into the physical origin of the large dielectric constants in such multiferroic composites. This is the first study to report superdielectric behavior in compounds related to either BNT-BT or SFO. Moreover, the dielectric constant is the highest among multiferroic composites at room temperature and among the highest in solid superdielectric materials.
In the second set of samples, SrFe12O19 – BNT-BT0.08 nanocomposites were investigated by X-ray diffraction, Impedance analyzer, Ferrotester and lock-in amplifier. The diffraction is confirming the involved phases. The dielectric constant values at low frequencies attained about 950, and relatively low dielectric losses are obtained, 0.12 at low frequencies. The electric and magnetoelectric behavior was described.
References
[1] M.M. Vopson, Fundamentals of Multiferroic Materials and Their Possible Applications, Critical Reviews in Solid State and Materials Sciences, 40 (2015) 223-250.
[2] Manipatruni S et al. 2018 Scalable energy-efficient magnetoelectric spin–orbit logic. Nature 565, 1–9. (doi:10.1038/s41586-018-0770-2)
[3] Nair M, Guduru R, Liang P, Hong J, Sagar V, Khizroev S. 2013 Externally controlled on-demand release of anti-HIV drug using magneto-electric nanoparticles as carriers. Nat. Commun. 4, 1707. (doi:10.1038/ncomms2717)
[4] Mushtaq F et al. 2019 Magnetoelectric 3D scaffolds for enhanced bone cell proliferation. Appl.
Mater. Today 16, 290–300. (doi:10.1016/j.apmt.2019.06.004)
[5] G. Flora, D. Gupta, A. Tiwari, Interdiscip Toxicol. 2012; Vol. 5(2): 47–58, doi: 10.2478/v10102-012-0009-2.
[6] R.C. Pullar, ACS Combinatorial Science 14 (2012) 425−433.
[7] Z. Donwantg, Y. Pu, P. Wang, 2015 Joint IEEE International Symposium on the Applications of Ferroelectric (ISAF), ISBN: 978-1-4799-9974-3, INSPEC Accession Number: 15330593.
[8] B. Want, M.D. Rather, R. Samad, Journal of Materials Science: Materials in Electronics 27 (2016) 5860–5866.
[9] H. Trivedi, V.V. Shvartsman, D.C. Lupascu, M.S.A. Medeiros, R.C. Pullar, A.L. Kholkin, P. Zelenovskiy, A. Sosnovskikh, V.Ya. Shurd, Nanoscale 7 (2015) 4489.
[10] J. Das, Y.-Y. Song, M. Wu, Journal of Applied Physics 108 (2010) 043911.
[11] M. Stingaciu, P.G. Reuvekamp, C.-W. Tai, R.K. Kremer, M. Johnsson, Journal of Materials Chemistry C 2 (2014) 325.
[12] D. Maurya, M. Peddigari, M.-G. Kang, L.D. Geng, N. Sharpes, V. Annapureddy, H. Palneedi, R. Sriramdas, Y. Yan, H.-C. Song, Y.U. Wang, J. Ryu, S. Priya, Journal of Materials Research 33 (2018) 2235-2263.
[13] J. Gao, D. Xue, W. Liu, C. Zhou, X. Ren, Actuators 6 (2017) 24.
[14] M. A. Subramanian, D. Li, N. Duan, B. A. Reisner, and A. W. Sleight, J. Solid State Chem. 151, 323 (2000).
[15] Z.-Y. Gao, Y.-P. Pu, J. Wei, Z.-J. Dong, and Y.-F. Cui, Phys. Status Solidi RRL 11, 1700142 (2017).
[16] M. Cernea, R. Radu, H. Amorín, S. G. Greculeasa, B. S. Vasile, V. A. Surdu, P. Ganea, R. Trusca, M. Hattab, C. Galassi, Nanomater. 10, 672 (2020).
[17] S. Fromille and J. Phillips, Mater. 7, 8197 (2014).
[18] R. D. Clark, Mater. 7, 2913 (2014).
[19] S. Guillemet-Fritsch, T. Lebey, M. Boulos, B. Durand, J. Europ. Ceram. Soc. 26, 1245 (2006).
[20] L. Marchin, S. Guillemet-Fritsch, B. Durand, A. A. Levchenko, A. Navrotsky, and T. Lebey, J. Am. Ceram. Soc. 91, 485 (2008).
[21] P. K. Patel, H. Singh, K. L. Yadav, Physica B 617, 413114 (2021).
[22] K. Lee, S. Hajra, M. Sahu, H. J. Kim, J. Alloy Compound 882, 160634 (2021).
[23] N. Bajpai, M. Saleem, and A. Mishra, J Mater Sci: Mater Electron 32, 12890 (2021).
[24] X. Liao, F. Peng, Y. Pu, S. Cao and D. Zhu, J. Electron. Mater. 50, 1963 (2021).
[25] X. W. Wang, B. K. Liang, Y. P. Zheng, S. N. Li, Y. F. Liang, Y. Q. Sun, Y. Y. Li, Y. C. Shi, B. H. Zhang, S. Y. Shang, J. Shang, Y. C. Hu, Physica B: Condensed Matter. 598, 412426 (2020).
[26] E. Yao, J. Li, W. Zou, L. Wang, S. Xu, T. Hu, L. Zhang, Z. Lu, G. Wang, D. Wang, F. Zhao, Ceram. Internat. 46, 23433 (2020).
[27] W. Hu, K. Lau, Y. Liu, R.L. Withers, H. Chen, L. Fu, B. Gong, and W. Hutchison, Chem. Mater. 27, 4934 (2015).
[28] M. Saleem, D. Singh, A. Mishra and D. Varshney, Mater. Res. Express 6, 026304 (2019).
[29] R. Andoulsi-Fezei, K. Horchani-Naifer & M. Férid, Eur. Phys. J. Plus 136, 791 (2021).
[30] R. Ahmed, R. Si, S. Rehman, Y. Yu, Q. Li, C. Wang, Physica B: Condensed Matter 603, 412704 (2021).
[31] M. Rekaby, Appl. Phys. A 126, 664 (2020).
[32] J. Phillips, Mater. 9, 918 (2016).
[33] N. Jenkins, C. Petty and J. Phillips, Mater. 9, 118 (2016).
[34] A. Singh, R. Chatterjee, S. K. Mishra, P. S. R. Krishna, and S. L. Chaplot, J. Appl. Phys. 111, 014113 (2012).
[35] Z. Xiong, Q. Liu, J. Tang, L. Fang, X. Zhang, J. Li, Y. Fu, J. Wang, Z. Gao and D. Shi, RSC Adv. 11, 14578 (2021).
[36] S.G. Greculeasa, C. Comanescu, N. Iacob, A. Kuncser, I. Smaranda, L. Amarande, M. Cioangher, M. Burdușel, V. Teodorescu, not published.
[37] L. Lutterotti, Acta Crystall. A Found. Crystall. 56(s1),
https://doi.org/10.1107/S0108767300021954.
[38] R.A. Brand, Phys. Res. B 28, 398 (1987).
[39] T. Takenaka, H. Nagata, J. Europ. Ceram. Soc. 25, 2693 (2005).
[40] T. Ghosh, S.K. Sharma, and D. Pradhan, ACS Sustainable Chem. Eng. 8 1445 (2020).
[41] C. Justin Raj, G. Paramesh, B. Shri Prakash, K.R.S. Preethi Meher, K.B.R. Varma, Mater. Res. Bull. 74, 1(2016).
[42] S. Ke, T. Li, M. Ye, P. Lin, W. Yuan, X. Zeng, L. Chen & H. Huang, Sci. Rep. 7, 10144 (2017).
[43] P. Ren, Z. Yang, W.G. Zhu, C.H.A. Huan, and L. Wang, J. Appl. Phys. 109, 074109 (2011).
[44] Z. Valdez-Nava, C. Cheballah, L. Laudebat, S. Guillemet-Fritsch, and T. Lebey, Conference Proceedings of TSEIM 2014, 14525150; DOI: 10.1109/ISEIM.2014.6870823.
[45] B. J. Evans, F. Grandjean, A. P. Lilot, R. H. Vogel, A. Gerard, J. Magn. Magn. Mater. 67, 123 (1987).
[46] M. Cernea, S.-G. Sandu, C. Galassi, R. Radu, V. Kuncser, J. Alloy. Compound. 561, 121 (2013).
[47] M. Cernea, S.–G. Greculeasa, R. Radu, G. Aldica, P. Ganea, V.A. Surdu, E. Tanasa (Vasile), M. Cioangher, N. Iacob, R. M. Costescu, J. Alloy. Compound. 831, 154850 (2020).
Dissemination of results
Published article:
1) Dana Gingasu, Ioana Mindru, Daniela C. Culita, Jose Maria Calderon‑Moreno, Cristina Bartha, Simona Greculeasa, Nicusor Iacob, Silviu Preda, Ovidiu Oprea, Applied Physics A (2021) 127:892.
Articles sent for publication:
1) S.G. Greculeasa, A.E Stanciu, A. Leca, A. Kuncser, L. Hrib, C. Chirila, I. Pasuk, V. Kuncser, Nanomaterials, second revision.
2) S.G. Greculeasa, C. Comanescu, N. Iacob, A. Kuncser, I. Smaranda, L. Amarande, M. Cioanger, M. Burdușel, V. Teodorescu, sent to Journal of Applied Physics.
3) Simona Greculeasa, Cezar Comanescu, Nicusor Iacob and Andrei Kuncser, sent to Romanian Reports in Physics.
4) S.G. Greculeasa, C. Comanescu, M. Cioangher, sent to Romanian Reports in Physics.
Article in preparation:
1) C. Bartha, C. Comanescu, S.G. Greculeasa, P. Badica, M. Grigoroscuta, A. Leca, A. Alexandru-Dinu, in the process of submission to Magnetochemistry.
Oral presentations:
1) S.G. Greculeasa, P. Palade, G. Schinteie, A. Leca, N. Iacob, A. Kuncser, F. Dumitrache, I. Lungu, G. Prodan, M. Cernea, R. Radu, R.M. Costescu, B.S. Vasile, V.A. Surdu, Roxana Trusca, H. Amorín, V. Kuncser, International Conference on the Applications of the Mössbauer Effect (ICAME), September 5th-10th, 2021, Brasov, Romania.
2) Simona Gabriela Greculeasa, Cezar Comanescu, Nicușor Iacob, Andrei Kuncser, Luminița Amarande, Marius Cioanger, Mihai Burdușel, Valentin Teodorescu, TIM 20-21, November 11th, 2021, virtual.
Poster presentation:
1) Cristina Bartha, Mihai Grigoroscuta, Simona Greculeasa, Nicusor Iacob, and Petre Badica, International Conference on the Applications of the Mössbauer Effect (ICAME), September 5th-10th, 2021, Brasov, Romania.
Participation without contribution:
1) Lindau Nobel Laureate Meeting, Hybrid: Lindau, Germany – virtual, June 27th – July 2nd, 2021.
PROJECTS/ NATIONAL PROJECTS
Copyright © 2025 National Institute of Materials Physics. All Rights Reserved